Extracellular Signal Molecules Bind to Specific Receptors
Yeast cells communicate with one another for mating by secreting a few kinds of small peptides. In contrast, cells in higher animals communicate by means of hundreds of kinds of signal molecules. These include proteins, small peptides, amino acids, nucleotides, steroids, retinoids, fatty acid derivatives, and even dissolved gases such as nitric oxide and carbon monoxide. Most of these signal molecules are secreted from the signaling cell into the extracellular space by exocytosis (discussed in Chapter 13). Others are released by diffusion through the plasma membrane, and some are exposed to the extracellular space while remaining tightly bound to the signaling cell's surface.
Regardless of the nature of the signal, the target cell responds by means of a specific protein called a receptor, which specifically binds the signal molecule and then initiates a response in the target cell. The extracellular signal molecules often act at very low concentrations (typically ≤ 10-8 M), and the receptors that recognize them usually bind them with high affinity (affinity constant Kα ≥ 108 liters/mole; see Figure 3-44). In most cases, these receptors are transmembrane proteins on the target cell surface. When they bind an extracellular signal molecule (a ligand),they become activated and generate a cascade of intracellular signals that alter the behavior of the cell. In other cases, the receptors are inside the target cell, and the signal molecule has to enter the cell to activate them: this requires that the signal molecules be sufficiently small and hydrophobic to diffuse across the plasma membrane (Figure 15-3).

Figure 15-3
The binding of extracellular signal molecules to either cell-surface receptors or intracellular receptors. Most signal molecules are hydrophilic and are therefore unable to cross the plasma membrane directly; instead, they bind to cell-surface receptors, (more...)
Extracellular Signal Molecules Can Act Over Either Short or Long Distances
Many signal molecules remain bound to the surface of the signaling cell and influence only cells that contact it (Figure 15-4A). Such contact-dependent signaling is especially important during development and in immune responses. In most cases, however, signal molecules are secreted. The secreted molecules may be carried far afield to act on distant targets, or they may act as local mediators, affecting only cells in the immediate environment of the signaling cell. This latter process is called paracrine signaling (Figure 15-4B). For paracrine signals to be delivered only to their proper target cells, the secreted molecules must not be allowed to diffuse too far; for this reason they are often rapidly taken up by neighboring target cells, destroyed by extracellular enzymes, or immobilized by theextracellular matrix.

Figure 15-4
Forms of intercellular signaling. (A) Contact-dependent signaling requires cells to be in direct membrane-membrane contact. (B) Paracrine signaling depends on signals that are released into the extracellular space and act locally on neighboring cells. (more...)
For a large, complex multicellular organism, short-range signaling is not sufficient on its own to coordinate the behavior of its cells. In these organisms, sets of specialized cells have evolved with a specific role in communication between widely separate parts of the body. The most sophisticated of these are nerve cells, or neurons, which typically extend long processes (axons) that enable them to contact target cells far away. When activated by signals from the environment or from other nerve cells, a neuron sends electrical impulses (action potentials) rapidly along itsaxon; when such an impulse reaches the end of the axon, it causes the nerve terminals located there to secrete a chemical signal called a neurotransmitter. These signals are secreted at specialized cell junctions called chemical synapses, which are designed to ensure that the neurotransmitter is delivered specifically to the postsynaptic target cell (Figure 15-4C). The details of this synaptic signaling process are discussed in Chapter 11.
A second type of specialized signaling cell that controls the behavior of the organism as a whole is an endocrine cell. These cells secrete their signal molecules, called hormones, into the bloodstream, which carries the signal to target cells distributed widely throughout the body (Figure 15-4D).
The mechanisms that allow endocrine cells and nerve cells to coordinate cell behavior in animals are compared inFigure 15-5. Because endocrine signaling relies on diffusion and blood flow, it is relatively slow. Synaptic signaling, by contrast, can be much faster, as well as more precise. Nerve cells can transmit information over long distances by electrical impulses that travel at rates of up to 100 meters per second; once released from a nerve terminal, aneurotransmitter has to diffuse less than 100 nm to the target cell, a process that takes less than a millisecond. Another difference between endocrine and synaptic signaling is that, whereas hormones are greatly diluted in the bloodstream and interstitial fluid and therefore must be able to act at very low concentrations (typically < 10-8 M), neurotransmitters are diluted much less and can achieve high local concentrations. The concentration of acetylcholinein the synaptic cleft of an active neuromuscular junction, for example, is about 5 × 10-4 M. Correspondingly, neurotransmitter receptors have a relatively low affinity for their ligand, which means that the neurotransmitter can dissociate rapidly from the receptor to terminate a response. Moreover, after its release from a nerve terminal, a neurotransmitter is quickly removed from the synaptic cleft, either by specific hydrolytic enzymes that destroy it or by specific membrane transport proteins that pump it back into either the nerve terminal or neighboring glial cells. Thus, synaptic signaling is much more precise than endocrine signaling, both in time and in space.

Figure 15-5
The contrast between endocrine and synaptic signaling. In complex animals, endocrine cells and nerve cells work together to coordinate the diverse activities of the billions of cells. Whereas different endocrine cells must use different hormones to communicate (more...)
The speed of a response to an extracellular signal depends not only on the mechanism of signal delivery, but also on the nature of the response in the target cell. Where the response requires only changes in proteins already present in the cell, it can occur in seconds or even milliseconds. When the response involves changes in gene expression and the synthesis of new proteins, however, it usually requires hours, irrespective of the mode of signal delivery.
Autocrine Signaling Can Coordinate Decisions by Groups of Identical Cells
All of the forms of signaling discussed so far allow one cell to influence another. Often, the signaling cell and target are different cell types. Cells, however, can also send signals to other cells of the same type, as well as to themselves. In such autocrine signaling, a cell secretes signal molecules that can bind back to its own receptors. Duringdevelopment, for example, once a cell has been directed along a particular pathway of differentiation, it may begin to secrete autocrine signals to itself that reinforce this developmental decision.
Autocrine signaling is most effective when performed simultaneously by neighboring cells of the same type, and it is likely to be used to encourage groups of identical cells to make the same developmental decisions. Thus, autocrine signaling is thought to be one possible mechanism underlying the "community effect” that is observed in earlydevelopment, during which a group of identical cells can respond to a differentiation-inducing signal but a single isolated cell of the same type cannot (Figure 15-6).

Figure 15-6
Autocrine signaling. A group of identical cells produces a higher concentration of a secreted signal than does a single cell. When this signal binds back to a receptor on the same cell type, it encourages the cells to respond coordinately as a group. (more...)
Unfortunately, cancer cells often use autocrine signaling to overcome the normal controls on cell proliferation and survival that we discuss later. By secreting signals that act back on the cell's own receptors, cancer cells can stimulate their own survival and proliferation and thereby survive and proliferate in places where normal cells of the same type could not. How this dangerous perturbation of normal cell behavior comes about is discussed in Chapter 23.
Gap Junctions Allow Signaling Information to Be Shared by Neighboring Cells
Another way to coordinate the activities of neighboring cells is through gap junctions. These are specialized cell-cell junctions that can form between closely apposed plasma membranes and directly connect the cytoplasms of the joined cells via narrow water-filled channels (see Figure 19-15). The channels allow the exchange of small intracellular signaling molecules (intracellular mediators), such as Ca2+ and cyclic AMP (discussed later), but not of macromolecules, such as proteins or nucleic acids. Thus, cells connected by gap junctions can communicate with each other directly, without having to surmount the barrier presented by the intervening plasma membranes (Figure 15-7).

Figure 15-7
Signaling via gap junctions. Cells connected by gap junctions share small molecules, including small intracellular signaling molecules, and can therefore respond to extracellular signals in a coordinated way.
As discussed in Chapter 19, the pattern of gap-junction connections in a tissue can be revealed either electrically, with intracellular electrodes, or visually, after the microinjection of small water-soluble dyes. Studies of this kind indicate that the cells in a developing embryo make and break gap-junction connections in specific and interesting patterns, strongly suggesting that these junctions have an important role in the signaling processes that occur between these cells. Mice and humans that are deficient in one particular gap-junction protein (connexin 43), for example, have severe defects in heart development. Like the autocrine signaling described above, gap-junction communication helps adjacent cells of a similar type to coordinate their behavior. It is still not known, however, which particular small molecules are important as carriers of signals through gap junctions, and the specific functions of gap-junction communication in animal development remain uncertain.
Each Cell Is Programmed to Respond to Specific Combinations of Extracellular Signal Molecules
A typical cell in a multicellular organism is exposed to hundreds of different signals in its environment. These signals can be soluble, bound to the extracellular matrix, or bound to the surface of a neighboring cell, and they can act in many millions of combinations. The cell must respond to this babel of signals selectively, according to its own specific character, which it has acquired through progressive cell specialization in the course of development. A cell may be programmed to respond to one combination of signals by differentiating, to another combination by multiplying, and to yet another by performing some specialized function such as contraction or secretion.
Most of the cells in a complex animal are also programmed to depend on a specific combination of signals simply to survive. When deprived of these signals (in a culture dish, for example), a cell activates a suicide program and kills itself--a process called programmed cell death, or apoptosis (Figure 15-8). Because different types of cells require different combinations of survival signals, each cell type is restricted to different environments in the body. The ability to undergo apoptosis is a fundamental property of animal cells, and it is discussed in Chapter 17.

Figure 15-8
An animal cell's dependence on multiple extracellular signals. Each cell type displays a set of receptors that enables it to respond to a corresponding set of signal molecules produced by other cells. These signal molecules work in combinations to regulate (more...)
In principle, the hundreds of signal molecules that animals make can be used to create an almost unlimited number of signaling combinations. The use of these combinations to control cell behavior enables an animal to control its cells in highly specific ways by using a limited diversity of signal molecules.
Different Cells Can Respond Differently to the Same Extracellular Signal Molecule
The specific way in which a cell reacts to its environment varies. It varies according to the set of receptor proteins the cell possesses, which determines the particular subset of signals it can respond to, and it varies according to the intracellular machinery by which the cell integrates and interprets the signals it receives (see Figure 15-1). Thus, a single signal molecule often has different effects on different target cells. The neurotransmitter acetylcholine, for example, stimulates the contraction of skeletal muscle cells, but it decreases the rate and force of contraction in heart muscle cells. This is because the acetylcholine receptor proteins on skeletal muscle cells are different from those on heart muscle cells. But receptor differences are not always the explanation for the different effects. In many cases, the same signal molecule binds to identical receptor proteins yet produces very different responses in different types of target cells, reflecting differences in the internal machinery to which the receptors are coupled (Figure 15-9).

Figure 15-9
Various responses induced by the neurotransmitter acetylcholine. Different cell types are specialized to respond to acetylcholine in different ways. (A and B) For these two cell types, acetylcholine binds to similar receptor proteins, but the intracellular (more...)
The Concentration of a Molecule Can Be Adjusted Quickly Only If the Lifetime of the Molecule Is Short
It is natural to think of signaling systems in terms of the changes produced when a signal is delivered. But it is just as important to consider what happens when a signal is withdrawn. During development, transient signals often produce lasting effects: they can trigger a change in the cell's development that persists indefinitely, through cell memory mechanisms such as those discussed in Chapters 7 and 21. In most cases in adult tissues, however, the response fades when a signal ceases. The effect is transitory because the signal exerts its effects by altering a set of molecules that are unstable, undergoing continual turnover. Thus, once the signal is shut off, the replacement of the old molecules by new ones wipes out all traces of its action. It follows that the speed with which a cell responds to signal removal depends on the rate of destruction, or turnover, of the molecules the signal affects.
It is also true, although much less obvious, that this turnover rate also determines the promptness of the response when a signal is turned on. Consider, for example, two intracellular signaling molecules X and Y, both of which are normally maintained at a concentration of 1000 molecules per cell. Molecule Y is synthesized and degraded at a rate of 100 molecules per second, with each molecule having an average lifetime of 10 seconds. Molecule X has a turnover rate that is 10 times slower than that of Y: it is both synthesized and degraded at a rate of 10 molecules per second, so that each molecule has an average lifetime in the cell of 100 seconds. If a signal acting on the cell boosts the rates of synthesis of both X and Y tenfold without any change in the molecular lifetimes, at the end of 1 second the concentration of Y will have increased by nearly 900 molecules per cell (10 × 100 - 100), while the concentration of X will have increased by only 90 molecules per cell. In fact, after a molecule's synthesis rate has been either increased or decreased abruptly, the time required for the molecule to shift halfway from its old to its new equilibriumconcentration is equal to its normal half-life--that is, equal to the time that would be required for its concentration to fall by half if all synthesis were stopped (Figure 15-10).

Figure 15-10
The importance of rapid turnover. The graphs show the predicted relative rates of change in the intracellular concentrations of molecules with differing turnover times when their synthesis rates are either (A) decreased or (B) increased suddenly by a (more...)
The same principles apply to proteins and small molecules, and to molecules in the extracellular space and inside cells. Many intracellular proteins have short half-lives, some surviving for less than 10 minutes. In most cases, these are proteins with key regulatory roles, whose concentrations are rapidly regulated in the cell by changes in their rates of synthesis. Likewise, any covalent modifications of proteins that occur as part of a rapid signaling process--most commonly, the addition of a phosphate group to an amino acid side chain--must be continuously removed at a rapid rate to make rapid signaling possible.
We shall discuss some of these molecular events in detail later for signaling pathways that operate via cell-surface receptors. But the principles apply quite generally, as the next example illustrates.
Nitric Oxide Gas Signals by Binding Directly to an Enzyme Inside the Target Cell
Although most extracellular signals are hydrophilic molecules that bind to receptors on the surface of the target cell, some signal molecules are hydrophobic enough and/or small enough to pass readily across the target-cell plasma membrane. Once inside, they directly regulate the activity of a specific intracellular protein. An important and remarkable example is the gas nitric oxide (NO), which acts as a signal molecule in both animals and plants. In mammals, one of its functions is to regulate smooth muscle contraction. Acetylcholine, for example, is released by autonomic nerves in the walls of a blood vessel, and it causes smooth muscle cells in the vessel wall to relax. Theacetylcholine acts indirectly by inducing the nearby endothelial cells to make and release NO, which then signals the underlying smooth muscle cells to relax. This effect of NO on blood vessels provides an explanation for the mechanism of action of nitroglycerine, which has been used for about 100 years to treat patients with angina (pain resulting from inadequate blood flow to the heart muscle). The nitroglycerine is converted to NO, which relaxes blood vessels. This reduces the workload on the heart and, as a consequence, it reduces the oxygen requirement of the heart muscle.
Many types of nerve cells use NO gas to signal to their neighbors. The NO released by autonomic nerves in the penis, for example, causes the local blood vessel dilation that is responsible for penile erection. NO is also produced as alocal mediator by activated macrophages and neutrophils to help them to kill invading microorganisms. In plants, NO is involved in the defensive responses to injury or infection.
NO gas is made by the deamination of the amino acid arginine, catalyzed by the enzyme NO synthase. Because it passes readily across membranes, dissolved NO rapidly diffuses out of the cell where it is produced and into neighboring cells. It acts only locally because it has a short half-life--about 5-10 seconds--in the extracellular space before it is converted to nitrates and nitrites by oxygen and water. In many target cells, including endothelial cells, NO binds to iron in the active site of the enzyme guanylyl cyclase, stimulating this enzyme to produce the small intracellular mediator cyclic GMP, which we discuss later (Figure 15-11). The effects of NO can occur within seconds, because the normal rate of turnover of cyclic GMP is high: a rapid degradation to GMP by a phosphodiesterase constantly balances the production of cyclic GMP from GTP by guanylyl cyclase. The drug Viagra inhibits this cyclic GMP phosphodiesterase in the penis, thereby increasing the amount of time that cyclic GMP levels remain elevated after NO production is induced by local nerve terminals. The cyclic GMP, in turn, keeps blood vessels relaxed and the penis erect.

Figure 15-11
The role of nitric oxide (NO) in smooth muscle relaxation in a blood vessel wall. Acetylcholine released by nerve terminals in the blood vessel wall activates NO synthase in endothelial cells lining the blood vessel, causing the endothelial cells to produce (more...)
Carbon monoxide (CO) is another gas that is used as an intercellular signal. It can act in the same way as NO, by stimulating guanylyl cyclase. These gases are not the only signal molecules that can pass directly across the target-cellplasma membrane. A group of small, hydrophobic, nongaseous hormones and local mediators also enter target cells in this way. But instead of binding to enzymes, they bind to intracellular receptor proteins that directly regulate genetranscription, as we discuss next.
Nuclear Receptors Are Ligand-activated Gene Regulatory Proteins
A number of small hydrophobic signal molecules diffuse directly across the plasma membrane of target cells and bind to intracellular receptor proteins. These signal molecules include steroid hormones, thyroid hormones, retinoids, andvitamin D. Although they differ greatly from one another in both chemical structure (Figure 15-12) and function, they all act by a similar mechanism. When these signal molecules bind to their receptor proteins, they activate the receptors, which bind to DNA to regulate the transcription of specific genes. The receptors are all structurally related, being part of the nuclear receptor superfamily. This very large superfamily also includes some receptor proteins that are activated by intracellular metabolites rather than by secreted signal molecules. Many family members have been identified by DNA sequencing only, and their ligand is not yet known; these proteins are therefore referred to asorphan nuclear receptors. The importance of such nuclear receptors in some animals is indicated by the fact that 1-2% of the genes in the nematode C. elegans code for them, although there are fewer than 50 in humans (see Figure 7-114).
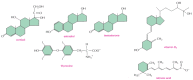
Figure 15-12
Some signaling molecules that bind to nuclear receptors. Note that all of them are small and hydrophobic. The active, hydroxylated form of vitamin D3 is shown. Estradiol and testosterone are steroid sex hormones.
Steroid hormones--which include cortisol, the steroid sex hormones, vitamin D (in vertebrates), and the moultinghormone ecdysone (in insects)--are all made from cholesterol. Cortisol is produced in the cortex of the adrenal glands and influences the metabolism of many types of cells. The steroid sex hormones are made in the testes and ovaries, and they are responsible for the secondary sex characteristics that distinguish males from females. Vitamin D is synthesized in the skin in response to sunlight; after it has been converted to its active form in the liver or kidneys, it regulates Ca2+ metabolism, promoting Ca2+ uptake in the gut and reducing its excretion in the kidneys. The thyroid hormones, which are made from the amino acid tyrosine, act to increase the metabolic rate in a wide variety of cell types, while the retinoids, such as retinoic acid, are made from vitamin A and have important roles as local mediators in vertebrate development. Although all of these signal molecules are relatively insoluble in water, they are made soluble for transport in the bloodstream and other extracellular fluids by binding to specific carrier proteins, from which they dissociate before entering a target cell (see Figure 15-3).
Beside a fundamental difference in the way they signal their target cells, most water-insoluble signal molecules differ from water-soluble ones in the length of time they persist in the bloodstream or tissue fluids. Most water-soluble hormones are removed and/or broken down within minutes of entering the blood, and local mediators and neurotransmitters are removed from the extracellular space even faster--within seconds or milliseconds. Steroid hormones, by contrast, persist in the blood for hours and thyroid hormones for days. Consequently, water-soluble signal molecules usually mediate responses of short duration, whereas water-insoluble ones tend to mediate responses that are longer lasting.
The intracellular receptors for the steroid and thyroid hormones, retinoids, and vitamin D all bind to specific DNAsequences adjacent to the genes the ligand regulates. Some receptors, such as those for cortisol, are located primarily in the cytosol and enter the nucleus after ligand binding; others, such as the thyroid and retinoid receptors, are bound to DNA in the nucleus even in the absence of ligand. In either case, the inactive receptors are bound to inhibitoryprotein complexes, and ligand binding alters the conformation of the receptor protein, causing the inhibitory complexto dissociate. The ligand binding also causes the receptor to bind to coactivator proteins that induce gene transcription (Figure 15-13). The transcriptional response usually takes place in successive steps: the direct activation of a small number of specific genes occurs within about 30 minutes and constitutes the primary response; the protein products of these genes in turn activate other genes to produce a delayed, secondary response; and so on. In this way, a simple hormonal trigger can cause a very complex change in the pattern of gene expression (Figure 15-14).

Figure 15-13
The nuclear receptor superfamily. All nuclear hormone receptors bind to DNA as either homodimers or heterodimers, but for simplicity we show them as monomers here. (A) The receptors all have a related structure. The short DNA-binding domain in each receptor (more...)

Figure 15-14
Responses induced by the activation of a nuclear hormone receptor. (A) Early primary response and (B) delayed secondary response. The figure shows the responses to a steroid hormone, but the same principles apply for all ligands that activate this family (more...)
The responses to steroid and thyroid hormones, vitamin D, and retinoids, like responses to extracellular signals in general, are determined as much by the nature of the target cell as by the nature of the signal molecule. Many types of cells have the identical intracellular receptor, but the set of genes that the receptor regulates is different in each cell type. This is because more than one type of gene regulatory protein generally must bind to a eucaryotic gene to activate its transcription. An intracellular receptor can therefore activate a gene only if there is the right combination of other gene regulatory proteins, and many of these are cell-type specific. Thus, each of these hormones induces a characteristic set of responses in an animal for two reasons. First, only certain types of cells have receptors for it. Second, each of these cell types contains a different combination of other cell-type-specific gene regulatory proteins that collaborate with the activated receptor to influence the transcription of specific sets of genes.
The molecular details of how nuclear receptors and other gene regulatory proteins control specific gene transcription are discussed in Chapter 7.
The Three Largest Classes of Cell-Surface Receptor Proteins Are Ion-Channel-linked, G-Protein-linked, and Enzyme-linked Receptors
As mentioned previously, all water-soluble signal molecules (including neurotransmitters and all signal proteins) bind to specific receptor proteins on the surface of the target cells that they influence. These cell-surface receptor proteins act as signal transducers. They convert an extracellular ligand-binding event into intracellular signals that alter the behavior of the target cell.
Most cell-surface receptor proteins belong to one of three classes, defined by the transduction mechanism they use.Ion-channel-linked receptors, also known as transmitter-gated ion channels or ionotropic receptors, are involved in rapid synaptic signaling between electrically excitable cells (Figure 15-15A). This type of signaling is mediated by a small number of neurotransmitters that transiently open or close an ion channel formed by the protein to which they bind, briefly changing the ion permeability of the plasma membrane and thereby the excitability of the postsynaptic cell. The ion-channel-linked receptors belong to a large family of homologous, multipass transmembrane proteins. Because they are discussed in detail in Chapter 11, we shall not consider them further here.

Figure 15-15
Three classes of cell-surface receptors. (A) Ion-channel-linked receptors, (B) G-protein-linked receptors, and (C) enzyme-linked receptors. Although many enzyme-linked receptors have intrinsic enzyme activity, as shown on the left, many others rely on (more...)
G-protein-linked receptors act indirectly to regulate the activity of a separate plasma-membrane-bound target protein, which can be either an enzyme or an ion channel. The interaction between the receptor and this target protein is mediated by a third protein, called a trimeric GTP-binding protein (G protein) (Figure 15-15B). The activation of the target protein can change the concentration of one or more intracellular mediators (if the target protein is an enzyme), or it can change the ion permeability of the plasma membrane (if the target protein is an ion channel). The intracellular mediators affected act in turn to alter the behavior of yet other signaling proteins in the cell. All of the G-protein-linked receptors belong to a large family of homologous, seven-pass transmembrane proteins.
Enzyme-linked receptors, when activated, either function directly as enzymes or are directly associated with enzymes that they activate (Figure 15-15C). They are formed by single-pass transmembrane proteins that have their ligand-binding site outside the cell and their catalytic or enzyme-binding site inside. Enzyme-linked receptors are heterogeneous in structure compared with the other two classes. The great majority, however, are protein kinases, or are associated with protein kinases, and ligand binding to them causes the phosphorylation of specific sets of proteins in the target cell.
There are some cell-surface receptors that do not fit into any of the above classes. Some of these depend on intracellular proteolytic events to signal the cell, and we discuss them only after we explain in detail how G-protein-linked receptors and enzyme-linked receptors operate. We start with some general principles of signaling via cell-surface receptors.
Most Activated Cell-Surface Receptors Relay Signals Via Small Molecules and a Network of Intracellular Signaling Proteins
Signals received at the surface of a cell by either G-protein-linked or enzyme-linked receptors are relayed into the cell interior by a combination of small and large intracellular signaling molecules. The resulting chain of intracellular signaling events ultimately alters target proteins, and these altered target proteins are responsible for modifying the behavior of the cell (see Figure 15-1).
The small intracellular signaling molecules are called small intracellular mediators, or second messengers (the "first messengers” being the extracellular signals). They are generated in large numbers in response to receptor activation and rapidly diffuse away from their source, broadcasting the signal to other parts of the cell. Some, such as cyclic AMP and Ca 2+ , are water-soluble and diffuse in the cytosol, while others, such as diacylglycerol, are lipid-soluble and diffuse in the plane of the plasma membrane. In either case, they pass the signal on by binding to and altering the behavior of selected signaling proteins or target proteins.
The large intracellular signaling molecules are intracellular signaling proteins. Many of these relay the signal into the cell by either activating the next signaling protein in the chain or generating small intracellular mediators. These proteins can be classified according to their particular function, although many fall into more than one category (Figure 15-16):

Figure 15-16
Different kinds of intracellular signaling proteins along a signaling pathway from a cell-surface receptor to the nucleus. In this example, a series of signaling proteins and small intracellular mediators relay the extracellular signal into the cell, (more...)
- 1.
Relay proteins simply pass the message to the next signaling component in the chain.
- 2.
Messenger proteins carry the signal from one part of the cell to another, such as from the cytosol to the nucleus.
- 3.
Adaptor proteins link one signaling protein to another, without themselves conveying a signal.
- 4.
Amplifier proteins, which are usually either enzymes or ion channels, greatly increase the signal they receive, either by producing large amounts of small intracellular mediators or by activating large numbers of downstream intracellular signaling proteins. When there are multiple amplification steps in a relay chain, the chain is often referred to as a signaling cascade.
- 5.
Transducer proteins convert the signal into a different form. The enzyme that makes cyclic AMP is an example: it both converts the signal and amplifies it, thus acting as both a transducer and an amplifier.
- 6.
Bifurcation proteins spread the signal from one signaling pathway to another.
- 7.
Integrator proteins receive signals from two or more signaling pathways and integrate them before relaying a signal onward.
- 8.
Latent gene regulatory proteins are activated at the cell surface by activated receptors and then migrate to thenucleus to stimulate gene transcription.
As shown in blue in Figure 15-16, other types of intracellular proteins also have important roles in intracellular signaling. Modulator proteins modify the activity of intracellular signaling proteins and thereby regulate the strength of signaling along the pathway. Anchoring proteins maintain specific signaling proteins at a precise location in the cell by tethering them to a membrane or the cytoskeleton. Scaffold proteins are adaptor and/or anchoring proteins that bind multiple signaling proteins together in a functional complex and often hold them at a specific location.
Some Intracellular Signaling Proteins Act as Molecular Switches
Many intracellular signaling proteins behave like molecular switches: on receipt of a signal they switch from an inactive to an active state, until another process switches them off. As we discussed earlier, the switching off is just as important as the switching on. If a signaling pathway is to recover after transmitting a signal so that it can be ready to transmit another, every activated molecule in the pathway must be returned to its original inactivated state.
The molecular switches fall into two main classes that operate in different ways, although in both cases it is the gain or loss of phosphate groups that determines whether the protein is active or inactive. The largest class consists of proteins that are activated or inactivated by phosphorylation (discussed in Chapter 3). For these proteins, the switch is thrown in one direction by a protein kinase, which adds one or more phosphate groups to the signaling protein, and in the other direction by a protein phosphatase, which removes the phosphate groups from the protein (Figure 15-17A). It is estimated that one-third of the proteins in a eucaryotic cell are phosphorylated at any given time.

Figure 15-17
Two types of intracellular signaling proteins that act as molecular switches. In both cases, a signaling protein is activated by the addition of a phosphate group and inactivated by the removal of the phosphate. (A) The phosphate is added covalently to (more...)
Many of the signaling proteins controlled by phosphorylation are themselves protein kinases, and these are often organized into phosphorylation cascades. One protein kinase, activated by phosphorylation, phosphorylates the next protein kinase in the sequence, and so on, relaying the signal onward and, in the process, amplifying it and sometimes spreading it to other signaling pathways. Two main types of protein kinases operate as intracellular signaling proteins. The great majority are serine/threonine kinases, which phosphorylate proteins on serines and (less often) threonines. Others are tyrosine kinases, which phosphorylate proteins on tyrosines. An occasional kinase can do both. Genome sequencing reveals that about 2% of our genes encode protein kinases, and it is thought that hundreds of distinct types of protein kinases are present in a typical mammalian cell.
The other main class of molecular switches involved in signaling are GTP-binding proteins (discussed in Chapter 3). These switch between an active state when GTP is bound and an inactive state when GDP is bound. Once activated, they have intrinsic GTPase activity and shut themselves off by hydrolyzing their bound GTP to GDP (Figure 15-17B). There are two major types of GTP-binding proteins--large trimeric GTP-binding proteins (also called G proteins),which relay the signals from G-protein-linked receptors (see Figure 15-15B), and small monomeric GTPases (also called monomeric GTP-binding proteins). The latter also help to relay intracellular signals, but in addition they are involved in regulating vesicular traffic and many other processes in eucaryotic cells.
As discussed earlier, complex cell behaviors, such as cell survival and cell proliferation, are generally stimulated by specific combinations of extracellular signals rather than by a single signal acting alone (see Figure 15-8). The cell therefore has to integrate the information coming from separate signals so as to make an appropriate response--to live or die, to divide or not, and so on. This integration usually depends on integrator proteins (see Figure 15-16), which are equivalent to the microprocessors in a computer: they require multiple signal inputs to produce an output that causes the desired biological effect. Two examples that show how such integrator proteins can operate are illustrated in Figure 15-18.

Figure 15-18
Signal integration. (A) Extracellular signals A and B both activate a different series of protein phosphorylations, each of which leads to the phosphorylation of protein Y but at different sites on the protein. Protein Y is activated only when both of (more...)
Intracellular Signaling Complexes Enhance the Speed, Efficiency, and Specificity of the Response
Even a single type of extracellular signal acting through a single type of G-protein-linked or enzyme-linked receptorusually activates multiple parallel signaling pathways and can thereby influence multiple aspects of cell behavior--such as shape, movement, metabolism, and gene expression. Indeed, these two main classes of cell-surface receptors often activate some of the same signaling pathways, and there is usually no obvious reason why a particular extracellular signal utilizes one class of receptors rather than the other.
The complexity of these signal-response systems, with multiple interacting relay chains of signaling proteins, is daunting. It is not clear how an individual cell manages to display specific responses to so many different extracellular signals, many of which bind to the same class of receptor and activate many of the same signaling pathways. One strategy that the cell uses to achieve specificity involves scaffold proteins (see Figure 15-16), which organize groups of interacting signaling proteins into signaling complexes (Figure 15-19A). Because the scaffold guides the interactions between the successive components in such a complex, the signal can be relayed with precision, speed, and efficiency; moreover, unwanted cross-talk between signaling pathways is avoided. In order to amplify a signal, however, and spread it to other parts of the cell, at least some of the components in most signaling pathways are likely to be freely diffusible.

Figure 15-19
Two types of intracellular signaling complexes. (A) A receptor and some of the intracellular signaling proteins it activates in sequence are preassembled into a signaling complex by a large scaffold protein. (B) A large signaling complex is assembled (more...)
In other cases, signaling complexes form only transiently, as when signaling proteins assemble around a receptor after an extracellular signal molecule has activated it. In some of these cases, the cytoplasmic tail of the activated receptor is phosphorylated during the activation process, and the phosphorylated amino acids then serve as docking sites for the assembly of other signaling proteins (Figure 15-19B). In yet other cases, receptor activation leads to the production of modified phospholipid molecules in the adjacent plasma membrane, and these lipids then recruit specific intracellular signaling proteins to this region of membrane. All such signaling complexes form only transiently and rapidly disassemble after the extracellular ligand dissociates from the receptor.
Interactions Between Intracellular Signaling Proteins Are Mediated by Modular Binding Domains
The assembly of both stable and transient signaling complexes depends on a variety of highly conserved, smallbinding domains that are found in many intracellular signaling proteins. Each of these compact protein modules binds to a particular structural motif in the protein (or lipid) with which the signaling protein interacts. Because of these modular domains, signaling proteins bind to one another in multiple combinations, like Lego bricks, with the proteins often forming a three-dimensional network of interactions that determines the route followed by the signaling pathway. By joining existing domains together in novel combinations, the use of such modular binding domains has presumably facilitated the rapid evolution of new signaling pathways.
Src homology 2 (SH2) domains and phosphotyrosine-binding (PTB) domains, for example, bind to phosphorylated tyrosines in a particular peptide sequence on activated receptors or intracellular signaling proteins. Src homology 3 (SH3) domains bind to a short proline-rich amino acid sequence. Pleckstrin homology (PH) domains (first described in the Pleckstrin protein in blood platelets) bind to the charged headgroups of specific phosphorylated inositol phospholipids that are produced in the plasma membrane in response to an extracellular signal; they thereby enable the protein they are part of to dock on the membrane and interact with other recruited signaling proteins. Some signaling proteins function only as adaptors to link two other proteins together in a signaling pathway, and they consist solely of two or more binding domains (Figure 15-20).

Figure 15-20
A hypothetical signaling pathway using modular binding domains. Signaling protein 1 contains three different binding domains, plus a catalytic protein kinase domain. It moves to the plasma membrane when extracellular signals lead to the creation of various (more...)
Scaffold proteins often contain multiple PDZ domains (originally found in a region of a synapse called the postsynaptic density), each of which binds to a specific motif on a receptor or signaling protein. The InaD scaffold protein in Drosophila photoreceptor cells is a striking example. It contains five PDZ domains, one of which binds a light-activated ion channel, while the others each bind to a different signaling protein involved in the response of the cell to light. If any of these PDZ domains are missing, the corresponding signaling protein fails to assemble in thecomplex, and the fly's vision is defective.
Some cell-surface receptors and intracellular signaling proteins are thought to cluster together transiently in specific microdomains in the lipid bilayer of the plasma membrane that are enriched in cholesterol and glycolipids. Some of the proteins are directed to these lipid rafts by covalently attached lipid molecules. Like scaffold proteins, these lipid scaffolds may promote speed and efficiency in the signaling process by serving as sites where signaling molecules can assemble and interact (see Figure 10-13).
Cells Can Respond Abruptly to a Gradually Increasing Concentration of an Extracellular Signal
Some cellular responses to extracellular signal molecules are smoothly graded in simple proportion to the concentration of the molecule. The primary responses to steroid hormones (see Figure 15-14) often follow this pattern, presumably because the nuclear hormone receptor protein binds a single molecule of hormone and each specific DNA recognition sequence in a steroid-hormone-responsive gene acts independently. As the concentration of hormone increases, the concentration of activated receptor-hormone complexes increases proportionally, as does the number of complexes bound to specific recognition sequences in the responsive genes; the response of the cell is therefore a gradual and linear one.
Many responses to extracellular signal molecules, however, begin more abruptly as the concentration of the moleculeincreases. Some may even occur in a nearly all-or-none manner, being undetectable below a threshold concentration of the molecule and then reaching a maximum as soon as this concentration is exceeded. What might be the molecular basis for such steep or even switchlike responses to graded signals?
One mechanism for sharpening the response is to require that more than one intracellular effector molecule orcomplex bind to some target macromolecule to induce a response. In some steroid-hormone-induced responses, for example, it seems that more than one activated receptor-hormone complex must bind simultaneously to specific regulatory sequences in the DNA to activate a particular gene. As a result, as the hormone concentration rises, gene activation begins more abruptly than it would if only one bound complex were sufficient for activation (Figure 15-21). A similar cooperative mechanism often operates in the signaling cascades activated by cell-surface receptors. As we discuss later, four molecules of the small intracellular mediator cyclic AMP, for example, must bind to each molecule of cyclic-AMP-dependent protein kinase to activate the kinase. Such responses become sharper as the number of cooperating molecules increases, and if the number is large enough, responses approaching the all-or-none type can be achieved (Figures 15-22 and 15-23).

Figure 15-21
The primary response of chick oviduct cells to the steroid sex hormone estradiol. When activated, estradiol receptors turn on the transcription of several genes. Dose-response curves for two of these genes are shown, one coding for the egg protein conalbumin (more...)

Figure 15-22
Activation curves as a function of signal-molecule concentration. The curves show how the sharpness of the response increases with an increase in the number of effector molecules that must bind simultaneously to activate a target macromolecule. The curves (more...)

Figure 15-23
One type of signaling mechanism expected to show a steep thresholdlike response. Here, the simultaneous binding of eight molecules of a signaling ligand to a set of eight protein subunits is required to form an active protein complex. The ability of the (more...)
Responses are also sharpened when an intracellular signaling molecule activates one enzyme and, at the same time, inhibits another enzyme that catalyzes the opposite reaction. A well-studied example of this common type of regulation is the stimulation of glycogen breakdown in skeletal muscle cells induced by the hormone adrenaline(epinephrine). Adrenaline's binding to a G-protein-linked cell-surface receptor leads to an increase in intracellular cyclic AMP concentration, which both activates an enzyme that promotes glycogen breakdown and inhibits an enzyme that promotes glycogen synthesis.
All of these mechanisms can produce responses that are very steep but, nevertheless, always smoothly graded according to the concentration of the extracellular signal molecule. Another mechanism, however, can produce true all-or-none responses, such that raising the signal above a critical threshold level trips a sudden switch in the responding cell. All-or-none threshold responses of this type generally depend on positive feedback; by this mechanism, nerve and muscle cells generate all-or-none action potentials in response to neurotransmitters (discussed in Chapter 11). The activation of ion-channel-linked acetylcholine receptors at a neuromuscular junction, for example, results in a net influx of Na+ that locally depolarizes the muscle plasma membrane. This causes voltage-gated Na+channels to open in the same membrane region, producing a further influx of Na+, which further depolarizes the membrane and thereby opens more Na+ channels. If the initial depolarization exceeds a certain threshold value, this positive feedback has an explosive "runaway” effect, producing an action potential that propagates to involve the entire muscle membrane.
An accelerating positive feedback mechanism can also operate through signaling proteins that are enzymes rather thanion channels. Suppose, for example, that a particular intracellular signaling ligand activates an enzyme located downstream in a signaling pathway and that two or more molecules of the product of the enzymatic reaction bind back to the same enzyme to activate it further (Figure 15-24). The consequence is a very low rate of synthesis of the product in the absence of the ligand. The rate increases slowly with the concentration of ligand until, at some threshold level of ligand, enough of the product has been synthesized to activate the enzyme in a self-accelerating, runaway fashion. The concentration of the product then suddenly increases to a much higher level. Through these and a number of other mechanisms not discussed here, the cell will often translate a gradual change in the concentration of a signaling ligand into a switchlike change, creating an all-or-none response by the cell.

Figure 15-24
An accelerating positive feedback mechanism. In this example, the initial binding of the signaling ligand activates the enzyme to generate a product that binds back to the enzyme, further increasing the enzyme's activity.
A Cell Can Remember The Effect of Some Signals
The effect of an extracellular signal on a target cell can, in some cases, persist well after the signal has disappeared. The enzymatic accelerating positive feedback system just described represents one type of mechanism that displays this kind of persistence. If such a system has been switched on by raising the concentration of intracellular activatingligand above threshold, it will generally remain switched on even when the extracellular signal disappears; instead of faithfully reflecting the current level of signal, the response system displays a memory. We shall encounter a specific example of this later, when we discuss a protein kinase that is activated by Ca2+ to phosphorylate itself and other proteins; the autophosphorylation keeps the kinase active long after Ca2+ levels return to normal, providing a memory trace of the initial signal.
Transient extracellular signals often induce much longer-term changes in cells during the development of a multicellular organism. Some of these changes can persist for the lifetime of the organism. They usually depend on self-activating memory mechanisms that operate further downstream in a signaling pathway, at the level of genetranscription. The signals that trigger muscle cell determination, for example, turn on a series of muscle-specific gene regulatory proteins that stimulate the transcription of their own genes, as well as genes producing many other muscle cell proteins. In this way, the decision to become a muscle cell is made permanent (see Figure 7-72B).
Cells Can Adjust Their Sensitivity to a Signal
In responding to many types of stimuli, cells and organisms are able to detect the same percentage of change in a signal over a very wide range of stimulus intensities. This requires that the target cells undergo a reversible process ofadaptation, or desensitization, whereby a prolonged exposure to a stimulus decreases the cells' response to that level of exposure. In chemical signaling, adaptation enables cells to respond to changes in the concentration of a signalingligand (rather than to the absolute concentration of the ligand) over a very wide range of ligand concentrations. The general principle is one of a negative feedback that operates with a delay. A strong response modifies the machinery for making that response, such that the machinery resets itself to an off position. Owing to the delay, however, a sudden change in the stimulus is able to make itself felt strongly for a short period before the negative feedback has time to kick in.
Desensitization to a signal molecule can occur in various ways. Ligand binding to cell-surface receptors, for example, may induce their endocytosis and temporary sequestration in endosomes. Such ligand-induced receptor endocytosis can lead to the destruction of the receptors in lysosomes, a process referred to as receptor down-regulation. In other cases, desensitization results from a rapid inactivation of the receptors--for example, as a result of a receptorphosphorylation that follows its activation, with a delay. Desensitization can also be caused by a change in a proteininvolved in transducing the signal or by the production of an inhibitor that blocks the transduction process (Figure 15-25).

Figure 15-25
Five ways in which target cells can become desensitized to a signal molecule. The inactivation mechanisms shown here for both the receptor and the intracellular signaling protein often involve phosphorylation of the protein that is inactivated, although (more...)
Having discussed some of the general principles of cell signaling, we now turn to the G-protein-linked receptors. These are by far the largest class of cell-surface receptors, and they mediate the responses to the great majority of extracellular signals. This superfamily of receptor proteins not only mediates intercellular communication; it is also central to vision, smell, and taste perception.
Summary
Each cell in a multicellular animal has been programmed during development to respond to a specific set of extracellular signals produced by other cells. These signals act in various combinations to regulate the behavior of the cell. Most of the signals mediate a form of signaling in which local mediators are secreted, but then are rapidly taken up, destroyed, or immobilized, so that they act only on neighboring cells. Other signals remain bound to the outer surface of the signaling cell and mediate contact-dependent signaling. Centralized control is exerted both by endocrine signaling, in which hormones secreted by endocrine cells are carried in the blood to target cells throughout the body, and by synaptic signaling, in which neurotransmitters secreted by nerve cell axons act locally on the postsynaptic cells that the axons contact.
Cell signaling requires not only extracellular signal molecules, but also a complementary set of receptor proteins in each cell that enable it to bind and respond to the signal molecules in a characteristic way. Some small hydrophobic signal molecules, including steroid and thyroid hormones, diffuse across the plasma membrane of the target cell and activate intracellular receptor proteins that directly regulate the transcription of specific genes. The dissolved gasesnitric oxide and carbon monoxide act as local mediators by diffusing across the plasma membrane of the target cell and activating an intracellular enzyme--usually guanylyl cyclase, which produces cyclic GMP in the target cell. But most extracellular signal molecules are hydrophilic and can activate receptor proteins only on the surface of the target cell; these receptors act as signal transducers, converting the extracellular binding event into intracellular signals that alter the behavior of the target cell.
There are three main families of cell-surface receptors, each of which transduces extracellular signals in a different way. Ion-channel-linked receptors are transmitter-gated ion channels that open or close briefly in response to the binding of a neurotransmitter. G-protein-linked receptors indirectly activate or inactivate plasma-membrane-bound enzymes or ion channels via trimeric GTP-binding proteins (G proteins). Enzyme-linked receptors either act directly as enzymes or are associated with enzymes; these enzymes are usually protein kinases that phosphorylate specific proteins in the target cell.
Once activated, enzyme- and G-protein-linked receptors relay a signal into the cell interior by activating chains of intracellular signaling proteins; some transduce, amplify, or spread the signal as they relay it, while others integrate signals from different signaling pathways. Many of these signaling proteins function as switches that are transiently activated by phosphorylation or GTP binding. Functional signaling complexes are often formed by means of modular binding domains in the signaling proteins; these domains allow complicated protein assemblies to function in signaling networks.
Target cells can use a variety of intracellular mechanisms to respond abruptly to a gradually increasing concentration of an extracellular signal or to convert a short-lasting signal into a long-lasting response. In addition, throughadaptation, they can often reversibly adjust their sensitivity to a signal to allow the cells to respond to changes in the concentration of a particular signal molecule over a large range of concentrations.